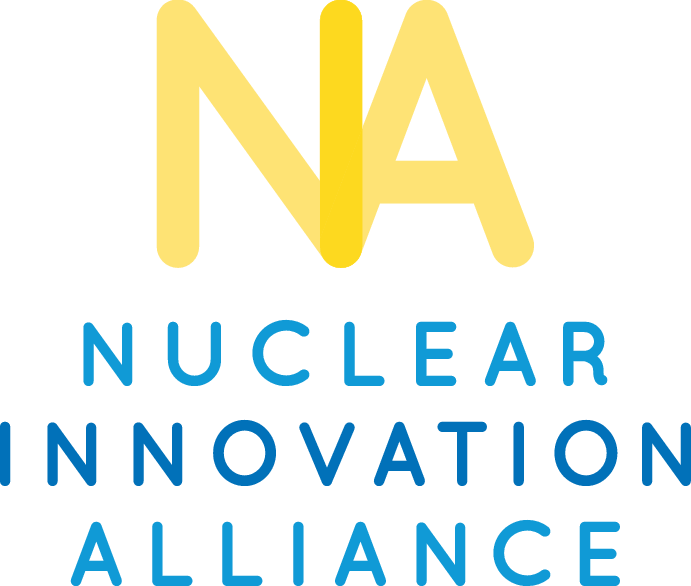
WASHINGTON, D.C. In the past three years, nine U.S. states enacted legislation to fully decarbonize the electricity sector by 2050 or sooner.[1] Three of those state laws require 85-100 percent reductions in economy-wide greenhouse gas emissions. At least 29 U.S. electric and gas utilities have pledged to reduce CO2 or GHG emissions by similar amounts.[2] Earlier this year, President Biden proposed a target of 100 percent clean electricity by 2035. Economic models that simulate decarbonization are indispensable to policymakers and utilities considering pathways to zero carbon emissions.[3] Those modelling tools inadequately characterize advanced nuclear technologies, a source of firm low-carbon power pivotal to deep decarbonization.
We reviewed 15 high-profile deep decarbonization modelling studies published since 2016 and assessed the characterization of advanced nuclear capabilities.[4] All such studies model decarbonization of 80 percent or more in the contiguous United States or in specific regions or states within the United States. Ten studies model decarbonization of the electric power sector and five model economy-wide decarbonization. 13 studies employ least-cost capacity expansion models, one study employs a “computable general equilibrium” (CGE) model, and one employs a scenario-based model without optimization.
We conclude that most models fall far short of characterizing the full range of advanced nuclear capabilities. Many advanced nuclear designs are capable of ramping and load-following. Certain designs include shut-off of smaller reactors within larger plants, thermal heat storage with molten salt, on-site battery storage, or hydrogen or other co-product production. Many advanced nuclear designs refuel less frequently and can operate at higher capacity factors than conventional designs. There are numerous advanced nuclear designs, with power ratings from 1.5 MWe to more than 1 GWe, that can be used in a variety of applications including district heating, co-generation, industrial heating, hydrogen production, desalination, and repowering idled plants.[5]
Just four studies model non-zero ramp rates for nuclear power plants and the remainder either assume a fixed power production profile or do not indicate otherwise. The four studies that model non-zero ramp rates assume maximum changes in reactor output of 0.04-0.42 percent of rated power per minute, compared to demonstrated ramp rates of 3-5 percent of rated power in modern commercial reactors in France, Germany, and other locations (Figure 1).[6] Some advanced nuclear designs may have even greater ramping and load-following capabilities than modern commercial reactors.
Seven studies assume average new nuclear capital costs of more than $6,000 / kW and only a single study assumes a capital cost lower than the average anticipated for commercial advanced nuclear designs (Figure 2).[7] Just four studies model modest annual reductions in the capital cost of new nuclear in the range of $17-81 / kW / year, short of expected capital cost reduction rates for advanced nuclear designs.[8]
A single study models the use of nuclear electric energy in industrial heating and two studies model the use of nuclear energy in hydrogen production. The remainder of the studies do not model the use of nuclear energy in district heating, co-generation, industrial heating, hydrogen production, desalination, or repowering. None of the studies model multiple nuclear designs despite a proliferation of designs.[9] Three studies permit power production from existing nuclear facilities but do not allow for the construction of new nuclear powerplants.
Most studies assume operational costs lower than those anticipated for commercial advanced nuclear designs (Figure 3). The studies assume total operational costs (fuel and non-fuel variable O&M) of $9-17 / kWh, compared to an anticipated range of $15-23 / kWh for commercial advanced reactors.[10] Nonetheless, none of the studies model intended reductions in variable or fixed O&M costs.[11] Annual operational costs for conventional and advanced nuclear designs are generally much lower than annual investment-related capital costs.
Figure 1. Nuclear Power Ramping Rates in Deep Decarbonization Modelling
Figure 2. Nuclear Power Capital Costs in Deep Decarbonization Modelling
Figure 3. Nuclear Power Variable O&M Costs in Deep Decarbonization Modelling
Many of the studies we reviewed rely on decarbonization models that directly inform energy policy, planning, and investment decisions. For example, the NREL ReEDS model was the primary analytical tool in the U.S. Department of Energy’s Sunshot, Wind Vision, Hydropower Vision, and GeoVision studies, each of which makes specific policy, regulatory, and investment recommendations related to renewable energy.[12] The E3 “PATHWAYS” model was used recently to support the California Energy Commission’s deep decarbonization study and Xcel Energy’s corporate climate strategy.[13] The EER EnergyPATHWAYS and RIO models were used to support the Massachusetts Office of Energy and Environmental Affairs’ decarbonization roadmap and Portland General Electric’s decarbonization study.[14]
A 2018 literature review concludes that deep decarbonization modeling scenarios that fairly characterize firm low-carbon generation resources—such as advanced nuclear energy—are more likely to yield lower overall decarbonization costs.[15] Our review reveals the exclusion or mischaracterization of advanced nuclear energy in 15 of the most prominent deep decarbonization studies published in recent years. It is incumbent upon the decarbonization research and modeling communities to fairly characterize advanced nuclear energy, and all such resources likely to contribute to decarbonization, given the widespread uses of such models in public policy and planning decisions.
For recommendations on how to solve this energy model issue, please refer to OnLocation's report published on our website: Analyzing the Role of Advanced Nuclear Energy in Deep Decarbonization: A Review of Market Opportunities and Modeling Challenges
Acknowledgement: Max Luke, Managing Consultant of Highland Energy Analytics was retained by the Nuclear Innovation Alliance to conduct this assessment of whether and how advanced nuclear capabilities are incorporated in recent deep decarbonization modelling studies.
[1] California (SB 100, signed into law September 2018); New Mexico (SB 489, March 2019); Nevada (SB 358, April 2019); Colorado (HB 1261, May 2019); Washington (SB 5116, May 2019); New York (S 6599, July 2019); Virginia (HB 1526, March 2020); Massachusetts (S 2995, January 2021); Oregon (HB 2021, July 2021).
[2] Clean Air Task Force, “State Technology-Inclusive Clean Energy Standards and Corporate Commitments – The National State of Play,” available at https://www.catf.us/wp-content/uploads/2020/10/State-Actions-and-Utility-Pledges-1.pdf (accessed 17 April 2021); The Climate Pledge, Signatories, available at https://www.theclimatepledge.com/us/en/Signatories.html (accessed 17 April 2021).
[3] One research analytics service reports that the annual number of publications that include the phrase “deep decarbonization” increased from an average of 113 in 2006-10 to 313 in 2011-15 and 1,471 in 2016-20. In 2020 there were 2,560 such publications, the greatest number of any year. See: Dimensions.ai, available at https://app.dimensions.ai/discover/publication (accessed 17 April 2021).
[4] We began with a broad review of deep decarbonization modeling studies and identified 80 such studies published since 2016. We identified the subset of studies that model deep decarbonization in the United States. Of those U.S.-focused studies, we identified the studies published in peer-reviewed academic journals with impact factors of 5.0 or higher or published by university centers or consultancies that focus on deep decarbonization research.
[5] See, for example: Kadak, A. C., “A Comparison of Advanced Nuclear Technologies,” Columbia University Center on Global Energy Policy, March 2017.
[6] Organisation for Economic Co-operation and Development (OECD) and Nuclear Energy Agency (NEA), “Technical and Economic Aspects of Load Following with Nuclear Power Plants,” June 2011; OECD and NEA, “Nuclear Energy and Renewables: System Effects in Low-carbon Electricity Systems,” 2020; Electric Power Research Institute (EPRI), “Program on Technology Innovation: Approach to Transition Nuclear Power Plants to Flexible Power Operations,” 2014.
[7] The Energy Options Network estimates a capital cost range of $2,217-6,323 / kW (2020 dollars) and an average of $4,085 based on information provided by eight advanced nuclear companies. See: Energy Innovation Reform Project (EIRP) and Energy Options Network (EON), “What Will Advanced Nuclear Power Plants Cost? A Standardized Cost Analysis of Advanced Nuclear Technologies in Commercial Deployment,” July 2017.
[8] Lovering, J. R. and J. R. McBride, “Chasing Cheap Nuclear: Economic Trade-Offs for Small Modular Reactors,” The Bridge, National Academy of Engineering, Fall 2020.
[9] Kadak, A. C., footnote 8.
[10] EIRP and EON, footnote 9.
[11] The U.S. Department of Energy Advanced Research Projects Agency-Energy (ARPA-E) funds a program that intends to drive a 10x reduction in advanced nuclear O&M costs. See: ARPA-E, “Generating Electricity Managed by Intelligent Nuclear Assets,” available at https://arpa-e.energy.gov/technologies/programs/gemina (accessed 29 April 2021).
[12] U.S. Department of Energy (DOE), “SunShot Vision Study,” February 2012; US DOE, “Wind Vision: A New Era for Wind Power in the United States,” March 2015; US DOE, “Hydropower Vision: A New Chapter for America’s 1st Renewable Electricity Source,” July 2016; US DOE, “GeoVision: Harnessing the Heat Beneath Our Feet,” May 2019.
[13] Energy+Environmental Economics (E3), “PATHWAYS Model,” available at https://www.ethree.com/tools/pathways-model/ (accessed 23 April 2021).
[14] Evolved Energy Research (EER), “Research,” available at https://www.evolved.energy/research (accessed 23 April 2021).
[15] Jenkins, J. D., Luke, M., & S. Thernstrom, “Getting to Zero Carbon Emissions in the Electric Power Sector,” Joule 2, 1-12 (2018).